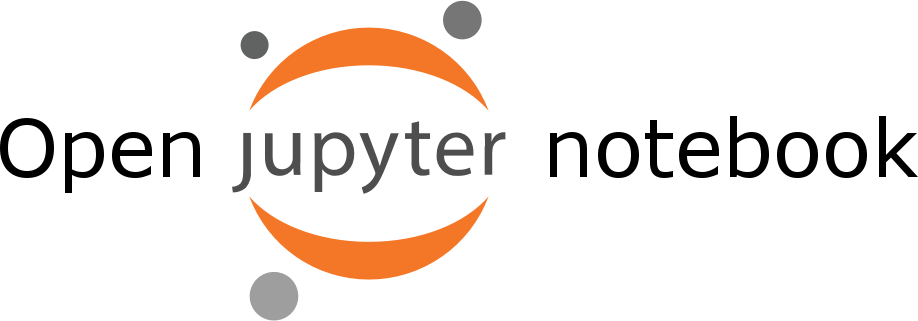
Risk Factor
Certain characteristic of economy (Inflation/GDP) or stock market itself (S&P 500)
Factor Model
Factor model uses movements in risk factors to explains portfolio returns
Questions which factor investing answers
- Why different asset have systematically lower or higher average returns?
- How to manage the asset portfolio with the underlying risks in mind?
- How to benefit of our ability to bear specific types of risks to generate returns?
Fama-French Model
Assumes linear relationship between empirical factors and stock returns:
- Market Factor (MER)
- Size Factor (SMB)
- Value Factor (HML)
- Profitability Factor (RMW)
- Investment Factor (CMA)
Factors are constructed daily from definitions, as illustrated previously
- They are global for the entire stock market
Factor sensitivities are calibrated using regression
- They represent “reward for taking a specific risk”, which is different for every stock
- Risk/Reward relationship is expected to hold over time
- Objective: maximize the model’s predictive power R2
Market Excess Return (MER)
- Market excess return (over RF rate) alone explains around 80% of asset movements
- Daily returns are ~normally distributed
- Relationship between returns of the overall market and returns of selected portfolio
Size (SMB) factor
- Small-cap companies typically bear additional risk premium - was it always the case?
- Python can help you to see that this factor has a different prevalence in different economic regimes
Value (HML) factor
- Value companies trade at higher yields to compensate for lack of growth potential
- Python can help you to see that this factor has different explanatory power in different market situations and on different portfolios (very interesting)
Profitability and investment factors
- Profitability factor (RMW) to attribute superior returns of companies with robust operating profit margins and strong competitive position among peers
- Investment factor (CMA) to segment companies based on their capital expenditures
- Analysts opinion: High capex structurally associated with growth companies, which puts usefulness of this factor in question
Evaluating 5-factor model
- Analyst opinion: High correlations between risk factors puts usefulness of 5-factor model into question.
- R2 10-20% for RMW, CMA
- 5 factor improvement only by 0.2%